James Olzmann
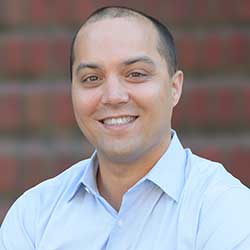
Professor of Molecular Therapeutics*
*and of Nutritional Sciences & Toxicology
Research Interests
The biology of cellular lipid homeostasis is a challenging frontier in science and medicine, and many outstanding questions remain. The importance of this field is underscored by the dysregulation of lipid metabolism in numerous diseases, including prevalent metabolic diseases, cancer, and degenerative diseases (e.g., neurodegeneration). Our lab exploits interdisciplinary strategies that integrate systems-level discovery methods (e.g. CRISPR-Cas9 screens and proteomics), chemical biology tools, and cell biology approaches to advance our understanding of the mechanisms that regulate lipid homeostasis. We are particularly interested in understanding the regulation of neutral lipid storage organelles called lipid droplets and the pathways that prevent oxidative lipid damage during ferroptosis. Our primary objectives include elucidating: 1) the cellular responses that combat lipotoxicity such as oxidative lipid damage and ferroptosis, 2) the regulation and functions of lipid droplets, 3) the mechanisms that govern lipid droplet proteome and lipidome composition and dynamics, and 4) the molecular underpinnings that mediate organelle crosstalk in lipid homeostasis. As we begin to uncover the molecular mechanisms the regulate lipid homeostasis, we strive to develop new therapeutics to target these pathways and improve human health. Ultimately, we aim to tackle difficult and complex problems in biology, catalyze progress, and translate our basic discoveries into novel therapeutics.
Current Projects
Lipotoxicity and ferroptosis
Lipotoxicity refers to the deleterious effects that lipids can have on cellular homeostasis and function. Accumulation of different types of lipids can induce distinct forms of lipotoxicity. For example, free fatty acids can induce ER stress by incorporation into ER lipids or can cause mitochondrial damage through incorporation into acylcarnitine. Some lipids play direct roles in cell death pathways. High levels of ceramide are associated with apoptotic signaling and the accumulation of oxidatively damaged phospholipids triggers the non-apoptotic cell death pathway known as ferroptosis. Ferroptosis has been implicated in the pathogenesis of a variety of degenerative conditions and targeted induction of ferroptosis is actively being pursued as a novel chemotherapeutic strategy to treat cancer.
Our current research aims to leverage genetic, cell biology, and biochemistry approaches to understand the mechanisms of ferroptosis and to discover the cellular factors that protect against these forms of damage. As we identify protective factors, we leverage chemical biology methods to develop small molecule inhibitors and activators as potential therapeutics. For example, we employed synthetic lethal CRISPR screens that led to the discovery of the oxidoreductase FSP1 as a new ferroptosis suppressor that generates the antioxidant form of CoQ to inhibit lipid peroxidation (Nature, 2019) and the discovery of LRP8 as a factor that enables cancer cell selenium scavenging to prevent ribosome stalling and disruptions in GPX4 translation (Nature Chem Biol, 2022). More recently, we developed small molecule inhibitors of FSP1 that sensitize cancer cells to ferroptosis (Cell Chem Biol, 2023).
Lipid droplet biogenesis and regulation
Lipid droplets are dynamic neutral lipid (i.e. fat and sterol esters) storage organelles that function as hubs of lipid metabolism, providing an “on demand” source of fatty acids that can be used for energy, membrane biogenesis, and lipid signaling pathways. Lipid droplets are formed at the endoplasmic reticulum (ER) in a process involving the deposition of neutral lipids between the leaflets of the ER, followed by the emergence of the lipid droplet into the cytoplasm from the outer leaflet. The mature lipid droplet can be degraded by lipolysis or through a selective autophagic pathway known as lipophagy. How these pathways are regulated under different conditions and in different cell types remain to be determined. Furthermore, alterations in lipid droplet abundance are associated with a wide variety of diseases. Indeed, the accumulation of large hepatic lipid droplets is the pathological hallmark of fatty liver disease. Interestingly, mutations in certain genes (e.g. TM6SF2 and PNPLA3) are associated with increased risk of fatty liver.
Our research aims to understand the mechanisms that regulate the biogenesis of lipid droplets and their functions in health and disease. For example, we discovered roles for lipid droplets in the prevention of lipotoxic damage to mitochondria (Dev Cell, 2017), developed chemoproteomic methods to study lipid droplet proteome remodeling (Dev Cell, 2018), and applied CRISPR screens to reveal new regulators of lipid droplet dynamics (Dev Cell, 2023; bioRxiv 2024). Some major questions include: How do lipid droplets form? How is the lipid and protein composition controlled? How are stored triacylglycerol molecules protected from oxidative damage? What are the functions of lipid droplets and how are these altered in diseases such as non-alcoholic fatty liver disease and Alzheimer's disease?
Small molecule tools and therapeutics
As we discover new mechanisms that impact lipid storage and lipotoxicity, our research seeks to translate these findings into meaningful tools and potential therapeutics. Through our chemistry collaborations, we employ small molecule screens and chemoproteomic approaches to identify hit compounds for development. For example, a recent high throughput small molecule screen in collaboration with Julia Schaletzky enabled the identification of structurally diverse FSP1 inhibitors that sensitize cancer cells to ferroptosis (Cell Chem Biol, 2023). In addition, chemoproteomic approaches with Dan Nomura have identified several compounds that impact cancer cell viability, including inhibitors of the ER shaping protein reticulon 4, focal adhesion kinase, and the regulator of cell growth and metabolism mTORC1. We have also contributed to the development of new E3 recruiters for targeted protein degradation. Current efforts focus on regulators of ferroptosis and new modalities of induced protein degradation.
Selected Publications
Mathiowetz, A., Meymand, E., Deol, K.D., Parlakgul, G., Lange, M., Pange, S.P., Roberts, M.A., Torres, E., Boone, C., Zhang, Y., Morgens, D.W., Tso, E., Zhou, Y., Talukdar, S., Levine, T.P., Ku, G., Arruda, A.P.#, Olzmann, J.A.# (2024) CLCC1 promotes nuclear pore complex assembly and hepatic neutral lipid flux. bioRxiv.
Mathiowetz, A. and Olzmann, J.A. (2024) Lipid droplets and cellular lipid flux. Nature Cell Biology. 26(3):331-345.
Dixon, S.J.# and Olzmann, J.A.# The cell biology of ferroptosis. (2024) Nature Reviews Molecular Cell Biology. 25(6):424-442.
Hendricks, J.M., Doubravsky, C.E., Wehri, E., Li, Z., Roberts, M.A., Deol, K.K., Lange, M., Lasheras-Otero, I., Momper, J.D., Dixon, S.J., Bersuker, K., Schlatetzky, J., Olzmann, J.A. (2023) Identification of structurally diverse FSP1 inhibitors that sensitize cancer cells to ferroptosis. Cell Chemical Biology. 30(9):1090-1103.e7.
Roberts, M.A., Deol, K.K., Lange, M., Leto, D., Mathiowetz, A.J., Stevenson, J., Hashemi, S.H., Morgens, D.W., Easter, E., Heydari, K., Nalls, M.A., Bassik, M.C., Kampmann, M., Kopito, R.R., Faghri, F., Olzmann, J.A. (2023) Parallel CRISPR-Cas9 screens reveal mechanisms of PLIN2 and lipid droplet regulation. Developmental Cell. 18:S1534-5807(23)00331-3.
Li, Z.*#, Lange, M.*, Dixon, S.J., and Olzmann, J.A.# (2023) Lipid quality control and ferroptosis: From concept to mechanism. Annual Review of Biochemistry. 93(1):499-528.
Li, Z., Ferguson, L., Deol, K.K., Roberts, M.A., Magtanong, L., Hendricks, J.M., Mousa, G.A., Kilinc, S., Schaefer, K., Wells, J.A., Bassik, M.C., Goga, A., Dixon, S.J., Ingolia, N., Olzmann, J.A. (2022) Ribosome stalling during selenoprotein translation exposes a ferroptosis vulnerability. Nature Chemical Biology. 18(7):751-761.
Bersuker, K., Hendricks, J., Li, Z., Magtanong, L., Ford, B., Tang, P.H., Roberts, M.A., Tong, B., Maimone, T.J., Zoncu, R., Nomura, D.K., Bassik, M.C., Dixon, S.J., Olzmann, J.A. (2019) The CoQ oxidoreductase FSP1 acts parallel to GPX4 to inhibit ferroptosis. Nature. 575(7784):688-692.
Olzmann, J.A. and Carvalho, P. (2019) Dynamics and functions of lipid droplets. Nature Review Cell Molecular Biology. 20(3): 137-155.
Bersuker, K., Peterson, C.W., To, M., Sahl, S.J., Savikhin, V., Grossman, E.A., Nomura, D.K., Olzmann, J.A. (2018) A proximity labeling strategy provides insights into the composition and dynamics of lipid droplet proteomes. Developmental Cell. 44, 97-112.
Nguyen, T.B., Louie, S.M., Daniele, J., Tran, Q., Dillin, A., Zoncu, R., Nomura, D.K., Olzmann, J.A. (2017) DGAT1-dependent lipid droplet biogenesis protects mitochondrial function during starvation-induced autophagy. Developmental Cell. 42, 9–21.
Last Updated 2024-08-25